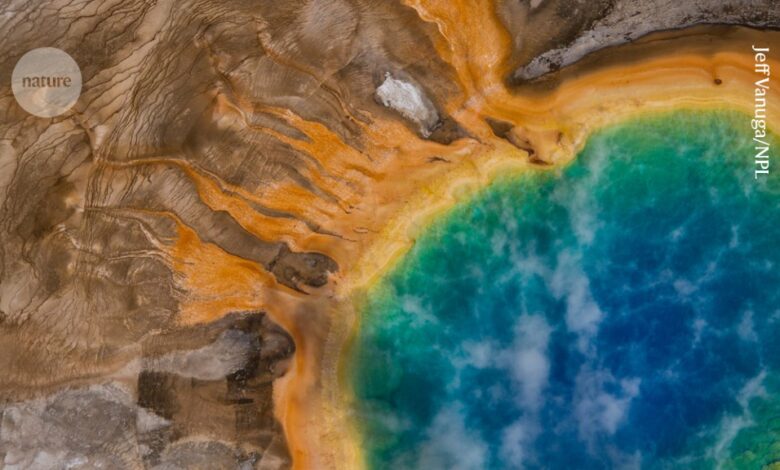
[ad_1]
The origin of life is one of the greatest challenges in science. It transcends conventional disciplinary boundaries, yet has been approached from within those confines for generations. Not surprisingly, these traditions have emphasized different aspects of the question.
Or rather, questions. The origin of life is really an extended continuum from the simplest prebiotic chemistry to the first reproducing cells, with molecular machines encoded by genes — machines such as ribosomes, the protein-building factories found in all cells. Most scientists agree that these nanomachines are a product of selection — but selection for what, where and how?
There is no consensus about what to look for, or where. Nor is there even agreement on whether all life must be carbon-based — although all known life on Earth is. Did meteorites deliver cells or organic material from outer space? Did life start on Earth in the hot waters of hydrothermal systems on land or in deep seas?
Observations alone cannot constrain these possibilities. The few geological traces that hint at early life are enigmatic. Is a bacterium-like imprint really a fossil, or some geochemical structure? Is a weak carbon isotope signature on the surface of a mineral a fingerprint of life (which accumulates the lighter carbon-12) or the result of another type of chemical activity?
Genes are not directly helpful either. Comparing gene sequences in modern organisms allows researchers to reconstruct a ‘tree of life’ going back to some of the earliest cells that have genes. Although the exact genetic make-up of this ancestral population is disputed, by definition it already had genes and proteins and so can tell us little about how they arose.
How did life begin? One key ingredient is coming into view
None of this precludes understanding the origin of life, but it does make competing hypotheses hard to prove or disprove unambiguously. Combine that with the overarching importance of the question and it’s clear why the field is beset with over-claims and counter-claims, which in turn warp funding, attention and recognition.
This context has splintered the field. Strongly opposed viewpoints have coexisted for decades over basic questions such as the source of energy and carbon, the need for light and whether selection acts on genes, chemical networks or cells.
To understand how life might have begun, researchers must stop cherry-picking the most beautiful bits of data or the most apparently convincing isolated steps, and explore the implications of these deep differences in context. Depending on the starting point, each hypothesis has different testable predictions. For example, if life started in a warm pond on land, the succession of steps leading from prebiotic chemistry to cells with genes is surprisingly different from those that must be posited if the first cells emerged in deep-sea hydrothermal vents.
Building coherent frameworks — in which all the steps in the continuum fit together — is essential to making real progress. To see why, here we highlight two of the most prominent frameworks, which propose radically distinct environments for the origin of life.
Prebiotic soup
Most people have heard of prebiotic soup. That’s in part because the hypothesis is grounded in the chemistry that works best for making many of the building blocks of living things. In the modern version of this idea, the synthesis of organic molecules begins with derivatives of cyanide, energized by ultraviolet radiation. This chemistry can produce relevant products, such as the nucleotide building blocks of genes, in high yields — although different reactions occur in distinct environments, ranging from laboratory equivalents of the atmosphere to geothermal ponds and streams1.
Where did all this cyanide come from? Meteorite impacts might be one source, but there is little agreement about that among geologists. Nor does this approach explain just how these “reservoirs of material … come to life when conditions change”2. That is, how compounds that formed under disparate conditions could persist for long periods (potentially millions of years) before somehow coming together and self-assembling into growing cells.
It’s time to admit that genes are not the blueprint for life
This framework posits that nucleotides are concentrated in a small pond. To form RNA, the simplest and most versatile genetic material, nucleotides must polymerize. That is most easily achieved by drying them out (polymerization is a type of dehydration reaction). Proponents imagine a succession of wet–dry cycles, in which the pond dries out to form polymers of RNA, then fills again with water containing more nucleotides and so on, cycle after cycle, making more and more RNA3.
But this concept raises some difficult questions. It places the onus on an ‘RNA world’, in which RNA acts both as a catalyst (in a similar way to enzymes) and as a genetic template that can be copied. The problems are that there is little evidence that RNA can catalyse many of the reactions attributed to it (such as those required for metabolism); and copying ‘naked’ RNA (that is not enclosed in compartments such as cells) favours the RNA strands that replicate the fastest. Far from building complexity, these tend to get smaller and simpler over time. Worse, by regularly drying everything out, wet–dry cycles keep forming random groupings of RNA (in effect, randomized genomes). The best combinations, which happen to encode multiple useful catalysts, are immediately lost again by re-randomization in the next generation, precluding the ‘vertical inheritance’ that is needed for evolution to build novelty.
If selection on RNA in drying ponds could somehow be made to generate greater complexity, what must it achieve? To make cells that grow and reproduce, RNA must encode metabolism: the network of hundreds of reactions that keeps all cells alive. Modern-day metabolic reactions bear no resemblance to the cyanide chemistry that makes nucleotides in this model. Evolution would therefore need to replace each and every step in metabolism, and there is no evidence that such a wholesale replacement is possible.
Unlike evolving an eye, a process in which intermediates have function, encoding only half the steps of a metabolic pathway (or half the pathways needed for a free-living cell) has little, if any, benefit. Can genes that encode multiple metabolic pathways have arisen at once? The odds against this are so great that the astrophysicist Fred Hoyle once compared it to a tornado blowing through a junkyard and assembling a jumbo jet. It is not good enough to counter that evolution will find a way: a real explanation needs to specify how.
On balance, we would say that prebiotic chemistry starting with cyanide can produce the building blocks of life, but most of the downstream steps predicted by this framework remain problematic.
Hydrothermal systems
Our own favoured scenario is that the chemistry of life reflects the conditions under which life began, in deep-sea hydrothermal systems on the early Earth4. In broad brush strokes, this means that gases such as carbon dioxide (the near-universal source of carbon in cells today) and hydrogen feed a network of reactions with a topology resembling metabolism. Genes and proteins arise within this spontaneous protometabolism and promote the flux of materials through the network, leading to cell growth and reproduction. There are plenty of problems here, too, but they differ from those in the prebiotic soup framework.
Origin of life theory involving RNA–protein hybrid gets new support
The first problem is that H2 and CO2 are not particularly reactive — indeed, their chemistry was largely ignored for decades, although rising interest in green chemistry is changing that. But deep-sea vents are labyrinths of interconnected pores, which have a topology resembling cells — acidic outside and alkaline inside. The flow of protons from the outside to the inside of these pores can drive work in much the same way that the inward flow of protons can drive CO2 fixation in cells today5. Research in the past few years shows that these conditions can drive the synthesis of carboxylic acids6 and long-chain fatty acids7, which can self-assemble into cell-like structures bounded by lipid bilayer membranes5.
But many chemists are troubled by the idea that, in the absence of enzymes to serve as catalysts, hydrothermal flow could drive scores of reactions through a network that prefigures metabolism, from CO2 right up to nucleotides. The chemist Leslie Orgel once dismissed this scenario as an “appeal to magic”. Certainly, further data are required, supporting or otherwise. Multiple steps have now been shown to occur spontaneously in core metabolic pathways (such as the Krebs cycle and amino-acid biosynthesis) without being driven by enzymes8, but this is still far from demonstrating flux through the entire network.
Polymerization is another stumbling block. Nucleotides have been polymerized in water on mineral surfaces9, but this raises similar questions to those noted for wet–dry cycles about how selection could act. If the problem is solved by polymerizing nucleotides inside growing protocells, mineral surfaces would not have been available. Polymerization would then have needed to happen in cell-like (aqueous gel) conditions, but without enzymes. If serious attempts to synthesize RNA under those conditions fail, the overall framework would need to be modified.
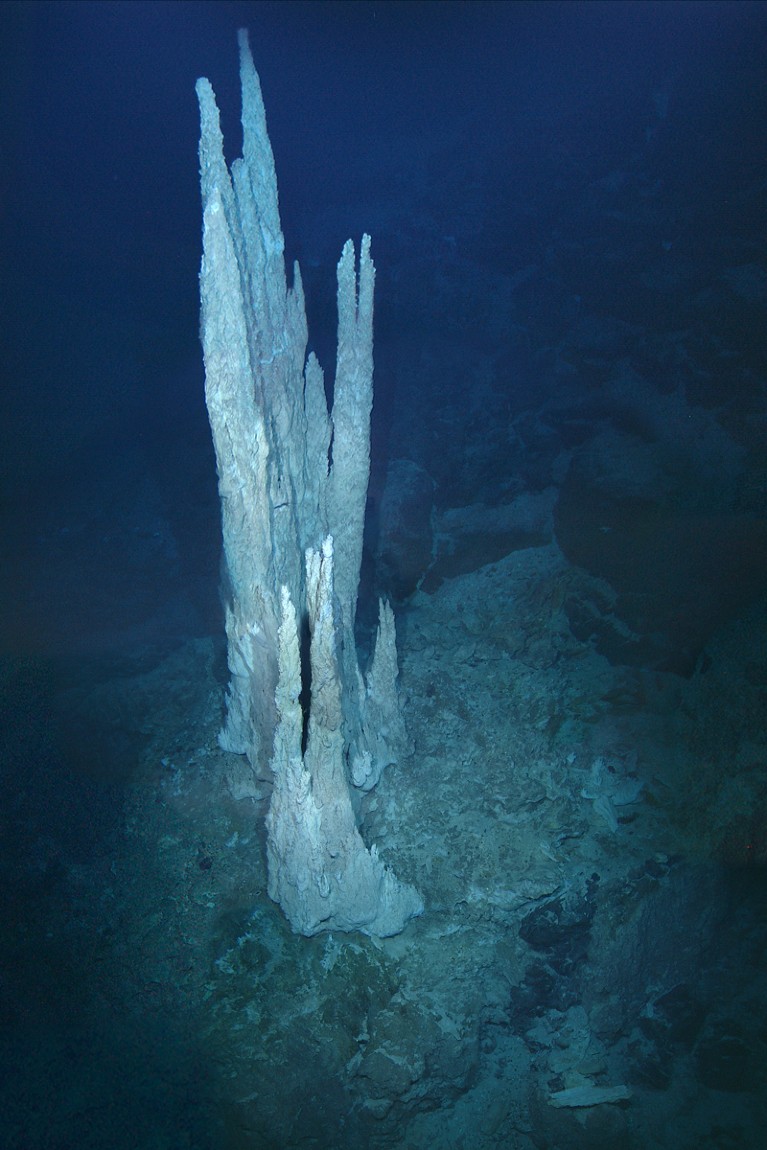
A 13-metre-tall carbonate chimney in the Lost City hydrothermal field in the Atlantic Ocean.Credit: Deborah Kelley and Mitch Elend, University of Washington
Conversely, if these difficult problems are resolved, then the hydrothermal scenario offers a promising route to the emergence of genetic information, overcoming Hoyle’s jumbo-jet argument. Patterns in the genetic code suggest direct physical interactions between amino acids and the nucleotides that encode them, especially for those formed most easily by metabolism5. Such associations mean that random RNA sequences could act as templates for non-random peptides that have a function in growing protocells. The first genes wouldn’t have had to encode metabolism, but just enhance flux through a spontaneous protometabolism — for example, by enabling the reaction between H2 and CO2.
Thus, in short, the two frameworks have different advantages and disadvantages, and it is premature to dismiss either.
Findings can be true but irrelevant
Similarly probing questions apply to other origins-of-life scenarios. If organic molecules were delivered from space — for instance, in carbonaceous chondrites such as the Murchison meteorite10 — then how and where did they come together, how did they polymerize, and so on? The delivery of organics from space simply stocks a soup and doesn’t solve most of the downstream problems — with the further issue that such a delivery method is unlikely to have been reliable and consistent at specific locations.
If life started out as droplets known as coacervates, in which immiscible liquids separate into distinct phases that promote different types of chemistry, then one must ask where all the precursors to feed their growth came from. And how did these phase-separated droplets morph into cells with different topology, in which these distinct chemistries now mostly occur under aqueous-gel conditions?
Prebiotic chemistry
Similar questions can be asked about ‘eutectic freezing’ (in which growing ice crystals concentrate the surrounding soup) and layered minerals or pores in volcanic rocks, such as basalt or floating pumice, that catalyse organic synthesis.
All of these fragments of scenarios are ‘true’, in that there is empirical evidence supporting each snapshot moment. But the fact that it is possible to make amino acids by passing electrical discharges through a Jovian mixture of gases, as the US chemist Stanley Miller famously did 70 years ago, does not mean that is how life began — merely that this chemistry is possible. Likewise, the fact that analogous chemistry can occur in hydrothermal systems, or from cyanide in terrestrial geothermal systems, or in interstellar space, does not mean that all of these environments were required for life to start, just that this chemistry is favoured under many conditions. The question is always: what happens next?
If none of these scenarios is ‘wrong’, then there is space in the field to pursue multiple frameworks. No one needs to abandon their favoured positions (yet). But brash claims for a breakthrough on the origin of life are unhelpful noise if they do not come in the context of a wider framework. The problem is ultimately answerable only if the whole question is taken seriously.
Look for convergence points
An important feature of these competing frameworks is that they must ultimately converge on cells with genes and proteins — on life as we know it on Earth. This convergence offers new possibilities for collaboration, because any answer will probably feature aspects of more than one framework. Exactly where these convergences occur will depend on which hypothetical steps are disproved.
Cofactors offer a possible convergence point. They got their name because they work together with an enzyme to catalyse a reaction. But from an origins-of-life perspective, the term is misleading because cofactors usually catalyse the same reaction on their own, albeit more slowly. Many cofactors derive from nucleotides, such as nicotinamide adenine dinucleotide. These might prove hard to make when starting with CO2. Could it be that cofactors were initially synthesized from cyanide, but, once in circulation, tended to catalyse CO2 chemistry, now driving a lifelike protometabolism that included their own synthesis11?
Bringing space rocks back to Earth could answer some of life’s biggest questions
Perhaps, but this idea also shows how important it is to test predictions within a specific framework first. In the simplest scenario, all of biochemistry begins from CO2 in a hydrothermal system, whereas the alternative scenario calls for at least two places and two types of chemistry — adding up to much more uncertainty. Occam’s razor says that the simplest scenario should be tested thoroughly first. If the simplest chemistry is shown not to work — that is, if it is not possible to synthesize cofactors from CO2 without cofactors — then the alternative can be taken seriously.
This question could be approached experimentally or using modern computational chemistry tools, but either way, the best way to make progress is to test the simplest idea to destruction first. If it can be shown not to work, then the convergence point might be real, and should be explored seriously.
Towards an answer
The origins-of-life field faces the same problems with culture and incentives that afflict all of science — overselling ideas towards publication and funding, too little common ground between competing groups and perhaps too much pride: too strong an attachment to favoured scenarios, and too little willingness to be proved wrong. These incentives are amplified by the difficulty of disproving complex interrelated hypotheses involving different disciplines when there is so little direct evidence — no ‘smoking gun’ to be discovered.
Changing this culture will take some work, given the political reality of science — the relentless pressure to publish, to secure funding, tenure or promotion — but it is necessary if the field wishes to continue attracting students. This requires that scientists, but also editors and funders, are aware of the issues that fragmented the field and work to overcome them. We highlight four priorities to begin to move in the right direction.
Train interdisciplinary scientists. Pursuing hypotheses across conventional disciplinary boundaries calls for a new generation of scientists — PhD students, postdoctoral researchers and early-career principal investigators (PIs) — with wide-ranging expertise and a willingness to test specific hypotheses within coherent wider frameworks. The field will clearly benefit from doctoral training that stresses collegiality, interdisciplinarity and the rigorous, open-minded testing of competing hypotheses.
Foster good communication. To promote such a culture, one of us (J.C.X.) co-founded the Origin of Life Early-career Network (OoLEN) in 2020, which has grown to more than 200 international researchers, from students to early-career PIs. It is run by volunteers and has no institutional ties, financial or otherwise. Members engage in debates through regular meetings (online or in-person), disseminate research and write articles together. There is still no shortage of disagreements, but that is part of scientific research and OoLEN promotes a healthy approach to them12.
For later-career researchers, conferences could help to reach across divides in similar ways. Physics meetings have provided examples. In one, proponents of loop quantum gravity and string theory switched sides in a debate, framing good-humoured but strong arguments against their own position in a constructive form of ‘steel manning’.
Embrace open science. Accepting that specific hypotheses will be disproved and that frameworks will be reshaped requires the publication of negative results — too often undervalued and unpublished. But it is clearly important for the field to know whether, for example, attempts to synthesize cofactors from CO2 fail — and, specifically, under what conditions.
Dissemination of negative data could be promoted in several ways. Most valuable is a more systematic use of open-access, community-driven knowledge bases that would host and curate data. These would help to collate experimental conditions, highlight genuine gaps in empirical evidence and enable analysis of large data sets through machine-learning studies.
Improve publishing practices. Researchers should aspire to contextualize their findings in cover letters, papers and press releases, to give a sense of how the work fits into a wider framework. Refraining from hype might seem unrealistic but could work if researchers implemented this practice in their roles as peer reviewers for papers and grants as well as authors.
Journal editors and grant-awarding bodies should also consider how polarized the field is to ensure fair reviews. One way to improve the peer-review process would be to enlist more early-career researchers, who tend to be less entrenched in their positions. Transparent peer review (in which anonymous reports are published with a paper) could also curb bias, because it enables constructive criticism without concealing prejudice.
It is too soon to aim for consensus or unity, and the question is too big; the field needs constructive disunity. Embracing multiple rigorous frameworks for the origin of life, as we advocate here, will promote objectivity, cooperation and falsifiability — good science — while still enabling researchers to focus on what they care most about. Without that, science loses its sparkle and creativity, never more important than here. With it, the field might one day get close to an answer.
Source link